DOI:
https://doi.org/10.14483/2256201X.20809Publicado:
01-01-2024Número:
Vol. 27 Núm. 1 (2024): Enero-junioSección:
Artículos de investigación científica y tecnológicaEfectos de la fertilización sobre la producción de hojarasca de bosques post-minería del Chocó Biogeográfico
Effects of Fertilization on Litter Production in Post-Mining Forests of the Biogeographic Chocó
Palabras clave:
Bosques tropicales, fósforo, limitación de nutrientes, nitrógeno, potasio, productividad primaria neta, sucesión vegetal (es).Palabras clave:
Tropical forests, phosphorus, nutrient limitation, nitrogen, potassium, net primary productivity, plant succession (en).Descargas
Referencias
Alvarado, A., & J. Raigosa. (2012). Nutrición y fertilización forestal en regiones tropicales (1ra ed. Universidad de Costa Rica, Asociación Costarricense de la Ciencia del Suelo.
Alvarez-Clare, S., M. C. Mack, & M. Brooks. (2013). A direct test of nitrogen and phosphorus limitation to net primary productivity in a lowland tropical wet forest. Ecology, 94(7), 1540-1551. https://doi.org/10.1890/12-2128.1 DOI: https://doi.org/10.1890/12-2128.1
Antonelli, A., & I. Sanmartin. (2011). Why are there so many plant species in the Neotropics? Taxon, 60 (2), 403-414. https://doi.org/10.1002/tax.602010 DOI: https://doi.org/10.1002/tax.602010
Barlow, J., T. A. Gardner, L. V. Ferreira, & C. A. Peres. (2007). Litter fall and decomposition in primary, secondary and plantation forests in the Brazilian Amazon. Forest Ecology and Management, 247(1-3), 91-97. https://doi.org/10.1016/j.foreco.2007.04.017 DOI: https://doi.org/10.1016/j.foreco.2007.04.017
Brown, S., & A. E. Lugo. (1990). Tropical secondary forests. Journal of Tropical Ecology, 6(1), 1-32. https://doi.org/10.1017/S0266467400003989 DOI: https://doi.org/10.1017/S0266467400003989
Campo, J., & Vázquez-Yanes, C. (2004). Effects of nutrient limitation on aboveground carbon dynamics during tropical dry forest regeneration in Yucatán, Mexico. Ecosystems, 7, 311-319. https://doi.org/10.1007/s10021-003-0249-2 DOI: https://doi.org/10.1007/s10021-003-0249-2
Campo, J., Solís, E., & Valencia, M. G. (2007). Litter N and P dynamics in two secondary tropical dry forests after relaxation of nutrient availability constraints. Forest Ecology and Management 252(1-3), 33-40. https://doi.org/10.1016/j.foreco.2007.06.022 DOI: https://doi.org/10.1016/j.foreco.2007.06.022
Celentano, D., Zahawi, R. A., Finegan, B., Ostertag, R., Cole, R. J., & Holl, K. D. (2011). Litterfall dynamics under different tropical forest restoration strategies in Costa Rica. Biotropica, 43(3), 279-287. https://doi.org/10.1111/j.1744-7429.2010.00688.x DOI: https://doi.org/10.1111/j.1744-7429.2010.00688.x
Chandra, J., & Keshavkant, S. (2021). Mechanisms underlying the phytotoxicity and genotoxicity of aluminum and their alleviation strategies: A review. Chemosphere, 278, 130384. https://doi.org/10.1016/j.chemosphere.2021.130384 DOI: https://doi.org/10.1016/j.chemosphere.2021.130384
Chapin III, F. S., Vitousek, P. M., & van Cleve K. (1986). The nature of nutrient limitation in plant communities. The American Naturalist, 127(1), 48-58. https://doi.org/10.1086/284466 DOI: https://doi.org/10.1086/284466
Chapin III, F. S., Woodwell, G. M., Randerson, J. T., Rastetter, E. B., Lovett, G. M., Baldocchi, D. D., Clark, D. A., Harmon, M. E., Schimel, D. S., Valentini, R., Wirth, C., Aber, J. D., Cole, J. J., Goulden, M. L., Harden, J. W., Heimann, M., Howarth, R. W., Matson, P. A., McGuire, A. D., … Schulze, E.-D. (2006). Reconciling carbon-cycle concepts, terminology, and methods. Ecosystems, 9, 1041-1050. https://doi.org/10.1007/s10021-005-0105-7 DOI: https://doi.org/10.1007/s10021-005-0105-7
Chave, J., Navarrete, D., Almeida, S., Álvarez, E., Aragão, L. E. O. C., Bonal, D., Châtelet, P., Silva-Espejo, J. E., Goret, J.-Y., von Hildebrand, P., Jiménez, E., Patiño, S., Peñuela, M. C., Phillips, O. L., Stevenson, P., & Malhi, Y. (2010). Regional and seasonal patterns of litterfall in tropical South America. Biogeosciences, 7, 43-55, https://doi.org/10.5194/bg-7-43-2010 DOI: https://doi.org/10.5194/bg-7-43-2010
Clark, D. A., Brown, S., Kicklighter, D. W., Chambers, J. D., Thomlinson, J. R., Ni, J., & Holland, E. (2001b). Net primary production in forest: an evaluation and synthesis of existing field data. Ecological Applications, 11(2), 371-384. https://doi.org/10.1890/1051-0761(2001)011[0371:NPPITF]2.0.CO;2 DOI: https://doi.org/10.1890/1051-0761(2001)011[0371:NPPITF]2.0.CO;2
Clark, D. A., Brown, S., Kicklighter, D. W., Chambers, J. D., Thomlinson, J. R. & Ni, J. (2001a). Measuring net primary production in forest: Concepts and field methods. Ecological Applications, 11(2), 356-370. https://doi.org/10.1890/1051-0761(2001)011[0356:MNPPIF]2.0.CO;2 DOI: https://doi.org/10.1890/1051-0761(2001)011[0356:MNPPIF]2.0.CO;2
Cleveland, C.C., Townsend, A. R., Schimel, D.S., Fisher, H., Howarth, R. W., Hedin, L. O., Perakis, S. S., Latty, E. F., von Fischer, J. C., Elseroad, A., & Wasson, M. F. (1999). Global patterns of terrestrial biological nitrogen (N2) fixation in natural ecosystems. Global Biogeochemical Cycles, 13(2), 623-645. https://doi.org/10.1029/1999GB900014 DOI: https://doi.org/10.1029/1999GB900014
Cleveland, C. C., Townsend, A. R., Taylor, P., Alvarez-Clare, S., Bustamante, M., Chuyong, G., Dobrowski, S. Z., Grierson, P., Harms, K. E., Houlton, B. Z., & Marklein, A., Parton, W., Porder, S., Reed, S. C., Sierra, C. A., Silver, W. L., Tammer. E. V. K., & Wieder, W. R. (2011). Relationships among net primary productivity, nutrients and climate in tropical rain forest: A pan-tropical analysis. Ecology Letter, 14(9), 939-947. https://onlinelibrary.wiley.com/doi/epdf/10.1111/j.1461-0248.2011.01658.x DOI: https://doi.org/10.1111/j.1461-0248.2011.01658.x
Cunha, H. F. V., Andersen, K. M., Lugli, L. F., Delgado Santana, F., Fonseca Aleixo, I., Martins Moraes, A., Garcia, S., Di Ponzio, R., Oblitas Mendoza, E., Brum, B., Schmeisk Rosa, J., Cordeiro, A. L., Tanaka Portela, B. T., Ribeiro, G., Deambrozi Coelho, S., Trierveiler de Souza, S., Siebert Silva, L., Antonieto, F., … Quesada, C. A. (2022). Direct evidence for phosphorus limitation on Amazon forest productivity. Nature, 608, 558-562. https://doi.org/10.1038/s41586-022-05085-2 DOI: https://doi.org/10.1038/s41586-022-05085-2
Cusack D. F., Silver, W. L., Torn, M. S., & McDowell, W. H. (2011). Effects of nitrogen additions on above and belowground carbon dynamics in two tropical forests. Biogeochemistry, 104, 203-225. https://doi.org/10.1007/s10533-010-9496-4 DOI: https://doi.org/10.1007/s10533-010-9496-4
Dantas, M., & Phillipson, J. (1989). Litterfall and litter nutrient content in primary and secondary Amazonian ‘terra firme’ rain forest. Journal of Tropical Ecology, 5(1), 27-36. https://doi.org/10.1017/S0266467400003199 DOI: https://doi.org/10.1017/S0266467400003199
Davidson, E. A., Reis de Carvalho, C. J., Figueira, A. M., Ishida, F. Y., Ometto, J. P. H. B., Nardoto, G. B., Sabá, R. T., Hayashi, S. N., Leal, E. C., Vieira, I. C. G., & Martinelli, L. A. (2007). Recuperation of nitrogen cycling in Amazonian forests following agricultural abandonment. Nature, 447, 995-998. https://doi.org/10.1038/nature05900 DOI: https://doi.org/10.1038/nature05900
Davidson, E.A., de Carvalho, C. J. R., Vieira, I. C. G., Figueiredo, R. D., Moutinho, P., Ishida, F. Y., dos Santos, M. T. P., Guerrero, J. B., Kalif, K., & Sabá, R. T. (2004). Nitrogen and phosphorus limitation of biomass growth in a tropical secondary forest. Ecological Applications, 14(SP4), 150-163. https://doi.org/10.1890/01-6006 DOI: https://doi.org/10.1890/01-6006
Del Grosso, S., Parton, W., Stohlgren, T., Zheng, D. L., Bachelet, D., Prince, S., Hibbard, K., & Olson, R. (2008). Global potential net primary production predicted from vegetation class, precipitation, and temperature. Ecology, 89, 2117-2126. https://doi.org/10.1890/07-0850.1 DOI: https://doi.org/10.1890/07-0850.1
FAO & PNUM. (2020). El estado de los bosques del mundo 2020. Los bosques, la biodiversidad y las personas. FAO. https://doi.org/10.4060/ca8642es DOI: https://doi.org/10.4060/ca8642es
Ferreira, M., Silva, J. L., Pereira, E. E., & Lamano-Ferreira, A. P. (2014). Litter fall production and decomposition in a fragment of secondary Atlantic Forest of São Paulo, sp, southeastern Brazil. Revista Árvore, 38(4), 591-600. https://doi.org/10.1590/S0100-67622014000400002 DOI: https://doi.org/10.1590/S0100-67622014000400002
Field C. B., Behrenfeld, M. J., Randerson, J. T., & Falkowski, P. (1998). Primary production of the biosphere: integrating terrestrial and oceanic components. Science, 281, 237-240. https://www.science.org/doi/10.1126/science.281.5374.237 DOI: https://doi.org/10.1126/science.281.5374.237
Harrington, R. A., Fownes, J. H., & Vitousek, P. M. (2001). Production and resource use efficiencies in N and P-limited tropical forests: A comparison of responses to long-term fertilization. Ecosystems, 4, 646-657. https://doi.org/10.1007/s10021-001-0034-z DOI: https://doi.org/10.1007/s10021-001-0034-z
Intergovernmental Panel on Climate Change (IPCC) (2014). Climate change 2014: Synthesis report. IPCC. https://www.ipcc.ch/report/ar5/syr/
Jiménez, E. M., Moreno, F. H., Lloyd, J., & Peñuela, M. C. (2009). Fine root dynamics for forests on contrasting soils in the Colombian Amazon. Biogeosciences, 6, 2809-2827. https://doi.org/10.5194/bg-6-2809-2009 DOI: https://doi.org/10.5194/bg-6-2809-2009
Kaspari, M., Garcia, M. N., Harms, K. E., Santana, M., Wright, S. J., & Yavitt, J. B. (2008). Multiple nutrients limit litterfall and decomposition in a tropical forest. Ecology Letters, 11, 35-43. https://doi.org/10.1111/j.1461-0248.2007.01124.x DOI: https://doi.org/10.1111/j.1461-0248.2007.01124.x
Lambers H., Chapin III, F. S., & Pons, T. L. (2008). Plant physiological ecology (2da ed.). Springer Science Business Media. https://doi.org/10.1007/978-0-387-78341-3 DOI: https://doi.org/10.1007/978-0-387-78341-3
LeBauer, D. S., & Treseder, K. K. (2008). Nitrogen limitation of net primary productivity in terrestrial ecosystems is globally distributed. Ecology, 89(2), 371-379. https://doi.org/10.1890/06-2057.1 DOI: https://doi.org/10.1890/06-2057.1
Loumeto, J. J. (2003). Litterfall and nutrient return in tropical rainforest in the Chaillu area (southwest Congo). Transactions on Ecology and the Environment, 64(1), 1237-1249. https://www.witpress.com/elibrary/wit-transactions-on-ecology-and-the-environment/64/1753
Mirmanto, E., Proctor, J., Green, J., Nagy, L., & Suriantata (1999). Effects of nitrogen and phosphorus fertilization in a lowland evergreen rainforest. Philosophical Transactions of the Royal Society B, 354, 1825-1829. https://doi.org/10.1098/rstb.1999.0524 DOI: https://doi.org/10.1098/rstb.1999.0524
Moreno, F., & Oberbauer, S. F. (2008). Dynamics of soil carbon in primary and secondary tropical forests in Colombia. En F. Bravo, V. LeMay, R. Jandl, & K. von Gadow (Eds.), Managing forest Ecosystems: The Challenge of Climate Change (pp. 283-296). Springer, Dordrecht. https://doi.org/10.1007/978-1-4020-8343-3_16 DOI: https://doi.org/10.1007/978-1-4020-8343-3_16
Osorio, N. W. (2014). Manejo de nutrientes en suelos del Trópico (2da ed.). Editorial L. Vieco.
Ostertag, R., Marín-Spiotta, E., Silver, W. L., & Schulten, J. (2008). Litterfall and decomposition in relation to soil carbon pools along a secondary forest chronosequence in Puerto Rico. Ecosystems, 11(5), 701-714. https://doi.org/10.1007/s10021-008-9152-1 DOI: https://doi.org/10.1007/s10021-008-9152-1
Pan, Y., Birdsey, R. A., Fang, J., Houghton, R., Kauppi, P. E., Kurz, W. A., Phillips, O. L., Shvidenko, A., Lewis, S. L., Canadell, J. G., Ciais, P., Jackson, R. B., Pacala, S. W., McGuire, A. D., Piao, S., Rautiainen, A., Stich, S., & Hayes, D. (2011). A large and persistent carbon sink in the world’s forests. Science, 333, 988-993. https://doi.org/10.1126/science.1201609 DOI: https://doi.org/10.1126/science.1201609
Paoli G., & Curran, L. (2007). Soil nutrients limit fine litter production and tree growth in mature lowland forest of southwestern Borneo. Ecosystems, 10, 503-518. https://doi.org/10.1007/s10021-007-9042-y DOI: https://doi.org/10.1007/s10021-007-9042-y
Paoli, G. D., Curran, L. M., & Zak, D. R. (2005). Phosphorus efficiency of aboveground productivity in Bornean rain forest: Evidence against the unimodal efficiency hypothesis. Ecology, 86,1548-1561. https://doi.org/10.1890/04-1126 DOI: https://doi.org/10.1890/04-1126
Poorter, L., Bongers, F., Aide, T. M., Almeyda, A. M., Balvanera, P., Becknell, J. M., Boukili, V., Brancalion, P. H. S., Broadbent, E. N., Chazdon, R. L., Craven, D., de Almeida-Cortez, J. S., Cabral, G. A. L., de Jong, B. H. J., Denslow, J. S., Dent, D. H., DeWalt, S. J., Dupuy, J. M., Durán, S. M. … Rozendaal, D. M. A. (2016). Biomass resilience of Neotropical secondary forests. Nature, 530, 211–214. https://doi.org/10.1038/nature16512 DOI: https://doi.org/10.1038/nature16512
Poveda, I. C., Rojas, C., Rudas, A., & Rangel, O. (2004). El Chocó biogeográfico: ambiente Físico. En O. Rangel (Ed.), Colombia Diversidad Biótica IV. El Chocó biogeográfico/Costa Pacífica. Instituto de Ciencias Naturales, Universidad Nacional de Colombia. https://repositorio.unal.edu.co/handle/unal/81941
Primack, R. B., & Vidal, O. (2019). Introducción a la biología de la conservación. Ediciones Científicas Universitarias.
Quinto, H., & Moreno, F. H. (2016). Precipitation effects on soil characteristics in tropical rain forests of the Chocó biogeographical region. Revista Facultad Nacional de Agronomía Medellín, 69, 7813-7823. http://dx.doi.org/10.15446/rfna.v69n1.54749 DOI: https://doi.org/10.15446/rfna.v69n1.54749
Quinto, H., Mena-Domínguez, Y., & Valoyes-Hinestroza, H. (2017). Relación entre la producción de hojarasca y las condiciones edáficas en bosques pluviales tropicales del Chocó Biogeográfico, Colombia. Actualidades Biológicas, 39 (106), 29-40. DOI: https://doi.org/10.17533/udea.acbi.v39n106a03
Quinto, H., L. Córdoba-Moreno, M., & Minotta-Moreno, N. N. (2019). Efectos de la fertilización del suelo sobre la producción de hojarasca en bosques pluviales tropicales del Chocó biogeográfico, Colombia. Bosque, 40(3), 315-322. http://doi.org/10.4067/S0717-92002019000300007 DOI: https://doi.org/10.4067/S0717-92002019000300315
Quinto, H., Ayala-Vivas, G., & Gutiérrez, H. (2022). Contenido de nutrientes, acidez y textura del suelo en áreas degradadas por la minería en el Chocó biogeográfico. Revista de la Academia Colombiana de Ciencias Exactas, Físicas y Naturales, 46(179), 514-528. https://doi.org/10.18257/raccefyn.1615 DOI: https://doi.org/10.18257/raccefyn.1615
Quinto, H., & Moreno, F. H. (2022). Influencia de los nutrientes del suelo sobre el crecimiento arbóreo en bosques del Pacífico colombiano. Colombia Forestal, 25(2), 30-44. https://doi.org/10.14483/2256201x.18232 DOI: https://doi.org/10.14483/2256201X.18232
Quinto, H., Cuesta-Nagles, J., Mosquera-Sánchez, I., Palacios-Hinestroza, L., & Peñaloza, H. (2013). Biomasa vegetal en zonas degradadas por minería en un bosque pluvial tropical del Chocó Biogeográfico. Revista Biodiversidad Neotropical, 3(1), 53-64. https://doi.org/10.18636/bioneotropical.v3i1.127 DOI: https://doi.org/10.18636/bioneotropical.v3i1.127
R Development Core Team (2012). A language and environment for statistical computing. R Foundation for Statistical Computing. https://www.r-project.org/
Ramírez, G., & Ledezma, E. (2007). Efectos de las actividades socio-económicas (minería y explotación maderera) sobre los bosques del departamento del Chocó. Revista Institucional Universidad Tecnológica del Chocó, 26, 58-65. https://dialnet.unirioja.es/servlet/articulo?codigo=2544441
Rodríguez, L. V. A. (1989). Consideraciones sobre la biomasa, composición química y dinámica del bosque pluvial tropical de colonias bajas. Bajo Calima, Buenaventura, Colombia. Convenio CONIF. http://bibliotecadigital.agronet.gov.co/bitstream/11348/6777/2/059.pdf
Salisbury, F. B., & Ross, C. W. (1994). Fisiología vegetal (4ta ed). Grupo Editorial Iberoamérica. https://apunteca.usal.edu.ar/id/eprint/2058/
Sansupa, C., Purahong, W., Wubet, T., Tiansawat, P., Pathom-Aree, W., & Teaumroong, N. (2021). Soil bacterial communities and their associated functions for forest restoration on a limestone mine in northern Thailand. PLoS ONE, 16(4), e0248806. https://doi.org/10.1371/journal.pone.0248806 DOI: https://doi.org/10.1371/journal.pone.0248806
Sayer, E. J., Wright, S. J., Tanner, E. V. J., Yavitt, J. B., Harms, K. E., Powers, J. S., & Kaspari, M., Garcia, M. N., & Turner, B. L. (2012). Variable responses of lowland tropical forest nutrient status to fertilization and litter manipulation. Ecosystems, 15, 387-400. https://doi.org/10.1007/s10021-011-9516-9 DOI: https://doi.org/10.1007/s10021-011-9516-9
Schuur, E. A. (2003). Productivity and global climate revisited: The sensitivity of tropical forest growth to precipitation. Ecology, 84, 1165-1170. https://doi.org/10.1890/0012-9658(2003)084[1165:PAGCRT]2.0.CO;2 DOI: https://doi.org/10.1890/0012-9658(2003)084[1165:PAGCRT]2.0.CO;2
Shetty, R., Vidya, C. S. N., Prakash, N. B., Lux, A., & Vaculik, M. (2021). Aluminum toxicity in plants and its possible mitigation in acid soils by biochar: A review. Science of the Total Environment, 765, 142744. https://doi.org/10.1016/j.scitotenv.2020.142744 DOI: https://doi.org/10.1016/j.scitotenv.2020.142744
Sullivan, B. W., Alvarez-Clare, S., Castle, S. C., Porder, S., Reed, S. C., Schreeg, L., Cleveland, C. C., & Townsend, A. R. (2014). Assessing nutrient limitation in complex forested ecosystems: alternatives to large-scale fertilization experiments. Ecology, 95(1), 668-681. https://doi.org/10.1890/13-0825.1 DOI: https://doi.org/10.1890/13-0825.1
Tierra Digna (2016). La minería en Chocó en clave de derechos. Investigación y propuestas para convertir la crisis socio-ambiental en paz y justicia territorial. Centro de Estudios para la Justicia Social (CEJS). https://justiciaambientalcolombia.org/reporte-la-mineria-en-choco/
Tripler, C. E., Kaushal, S. S., Likens, G. E., & Walter, M. T. (2006). Patterns in potassium dynamics in forest ecosystems. Ecology Letters, 9, 451-466. https://doi.org/10.1111/j.1461-0248.2006.00891.x DOI: https://doi.org/10.1111/j.1461-0248.2006.00891.x
Valois, H., & Martínez-Ruiz, C. (2016). Vulnerability of native forests in the Colombian Chocó: Mining and biodiversity conservation. Bosque 37(2), 295-305. https://www.revistabosque.org/index.php/bosque/article/view/486 DOI: https://doi.org/10.4067/S0717-92002016000200008
Veldkamp, E., Schmidt, M., Powers, J. S., & Corre, M. D. (2020). Deforestation and reforestation impacts on soils in the Tropics. Nature Reviews Earth and Environment, 1(11), 590-605. https://doi.org/10.1038/s43017-020-0091-5 DOI: https://doi.org/10.1038/s43017-020-0091-5
Vitousek, P. M. (1984). Litterfall, nutrient cycling and nutrient limitation in tropical forests. Ecology, 65, 285-298. https://doi.org/10.2307/1939481 DOI: https://doi.org/10.2307/1939481
Vitousek, P. M., & Farrington, H. (1997). Nutrient limitation and soil development: Experimental test of a biogeochemical theory. Biogeochemistry, 37, 63-75. https://doi.org/10.1023/A:1005757218475 DOI: https://doi.org/10.1023/A:1005757218475
Vitousek, P. M., & Sanford Jr., R. L. (1986). Nutrient cycling in moist tropical forest. Annual Review of Ecology and Systematics, 17, 137-167. https://doi.org/10.1146/annurev.es.17.110186.001033 DOI: https://doi.org/10.1146/annurev.ecolsys.17.1.137
Vitousek, P. M., Menge, D. N. L., Reed, S. C., & Cleveland C. C. (2013). Biological nitrogen fixation: Rates, patterns and ecological controls in terrestrial ecosystems. Philosophical Transactions of the Royal Society B, 368, 20130119. http://dx.doi.org/10.1098/rstb.2013.0119 DOI: https://doi.org/10.1098/rstb.2013.0119
Walker, L. R. (1993). Nitrogen fixers and species replacements in primary succession. En J. Miles & D. W. H. Walton (Eds.), Primary Succession on Land (pp. 249-272). Blackwell.
Walker L. R., & del Moral, R. (2008). Lessons from primary succession for restoration of severely damaged habitats. Applied Vegetation Science, 12, 55-67. https://doi.org/10.1111/j.1654-109X.2009.01002.x DOI: https://doi.org/10.1111/j.1654-109X.2009.01002.x
Walker, T. W., & Syers, J. K. (1976). The fate of phosphorus during pedogenesis. Geoderma, 15, 1-19. https://doi.org/10.1016/0016-7061(76)90066-5 DOI: https://doi.org/10.1016/0016-7061(76)90066-5
Wright, J., Yavitt, J., Wurzburger, N., Turner, B., Tanner, E., Sayer, E., Santiago, L., Kaspari, M., Hedin, L., Harms, K., García, M., & Corre, M. (2011). Potassium, phosphorus, or nitrogen limit root allocation, tree growth, or litter production in a lowland tropical forest. Ecology, 92(8), 1616-1625. https://doi.org/10.1890/10-1558.1 DOI: https://doi.org/10.1890/10-1558.1
Wright, S. J., Turner, B. L., Yavitt, J. B., Harms, K. E., Kaspari, M., Tanner, E. V. J., Bujan, J., Griffin, E. A., Mayor, J. R., Pasquini, S. C., Sheldrake, M., & Garcia, M. N. (2018). Plant responses to fertilization experiments in lowland, species-rich, tropical forests. Ecology, 99(5), 1-10. https://doi.org/10.1002/ecy.2193 DOI: https://doi.org/10.1002/ecy.2193
Zhu, X., Jiang, X., Singh, A. K., Zeng, H., Chen, C., Lu, E. & Liu, W. (2022). Reduced litterfall and decomposition alters nutrient cycling following conversion of tropical natural forests to rubber plantations. Ecological Indicators, 138, 108819. https://doi.org/10.1016/j.ecolind.2022.108819. DOI: https://doi.org/10.1016/j.ecolind.2022.108819
Tabla 1. Características estructurales, ecológicas y edáficas de los bosques maduros y bosques post-minería de 30-35 años en Jigualito, Chocó Biogeográfico, Colombia.
Cómo citar
APA
ACM
ACS
ABNT
Chicago
Harvard
IEEE
MLA
Turabian
Vancouver
Descargar cita
Visitas
Dimensions
PlumX
Descargas
Recibido: 27 de abril de 2023; Aceptado: 20 de noviembre de 2023
Resumen
Los nutrientes del suelo son considerados como factores limitantes de la productividad primaria neta (PPN) de los bosques tropicales. En este sentido, se ha planteado que, en etapas sucesionales iniciales, la PPN está limitada por N y, en etapas tardías, por P. Para probar dicha hipótesis, se midió la producción de hojarasca y el contenido de nutrientes en cinco tratamientos de fertilización (control, N, P, K y NPK) en bosques maduros y de post-minería del Chocó Biogeográfico. Se determinó que la producción de hojarasca post-minería fue mayor con la aplicación de N, K, y NPK, mientras que, en bosques maduros, fue mayor con la aplicación de N. Además, se registró un efecto significativo de la aplicación de N sobre el contenido foliar de N en post-minería. En conclusión, se evidenció una limitación nutricional múltiple de la PPN en etapas sucesionales tempranas, lo que denota un cambio en la limitación con la sucesión.
Palabras clave:
bosques tropicales, fósforo, limitación de nutrientes, nitrógeno, potasio, productividad primaria neta, sucesión vegetal.Abstract
Soil nutrients are regarded as limiting factors of the net primary productivity (PPN) of tropical forests. In this sense, it has been suggested that, in initial successional stages, the PPN is limited by N, as well as by P in late stages. To test this hypothesis, litter production and nutrient content were measured for five fertilization treatments (control, N, P, K, and NPK) in mature and post-mining forests of the Biogeographic Chocó. It was determined that the post-mining litter production was higher with the application of N, K, and NPK, whereas, in mature forests, it was higher with the application of N. In addition, a significant effect of the application of N on the post-mining foliar N content was recorded. In conclusion, a multiple nutritional limitation of the PPN was evidenced in early successional stages, which denotes a change in the limitation with succession.
Keywords:
tropical forests, phosphorus, nutrient limitation, nitrogen, potassium, net primary productivity, plant succession.INTRODUCCIÓN
El bosque tropical es considerado uno de los ecosistemas terrestres más importantes del mundo debido a su extensión geográfica, complejidad ecológica, biodiversidad y endemismo (Antonelli & Sanmartin, 2011), así como a altas tasas de productividad primaria neta (PPN) (Pan et al., 2011). Particularmente, la alta PPN de estos bosques tiene una relevancia adicional, pues representa más del 36 % de la PPN global (Field et al., 1998), lo cual corrobora su función como sumideros y reservorios de carbono y su papel en la mitigación del cambio climático global (Clark et al., 2001a; Pan et al., 2011; IPCC, 2014). Sin embargo, para comprender plenamente la función de estos ecosistemas en la mitigación del calentamiento global, es necesario evaluar la influencia de factores ambientales y biológicos sobre dicha PPN. Diversos estudios muestran que la PPN está determinada por factores como precipitación, radiación solar, temperatura, tipos de suelos, nutrientes, estadio sucesional, diversidad, estructura y composición (Schuur, 2003; Del Grosso et al., 2008; Jiménez et al., 2009; Cleveland et al., 2011; Cunha et al., 2022). Por ello, se ha observado un amplio rango de variación en los trópicos, i.e., de entre 1.2 y 15.2 Mg.ha-1.año-1 (Clark et al., 2001b). Particularmente, se ha evidenciado que los nutrientes del suelo están entre los elementos que más restringen la PPN (Vitousek, 1984; Chapin III et al., 1986; Lamber et al., 2008). En especial, los contenidos de nitrógeno (N), fósforo (P) y potasio (K) suelen ser los más limitantes (LeBauer & Treseder, 2008; Wright et al., 2011, 2018; Cunha et al., 2022).
Se ha planteado la hipótesis de que, en suelos tropicales en etapas sucesionales iniciales, hay una limitación por N, que se va mitigando con el paso del tiempo debido a la colonización de plantas capaces de fijar N2 atmosférico en simbiosis con bacterias (Walker ,1993). A su vez, esta fijación de N está limitada por la disponibilidad de P y la humedad. Por lo tanto, en la medida en que se presente una mayor colonización de plantas fijadoras de N2 y avance la sucesión, se reduciría tal limitación en el ecosistema (Cleveland et al., 1999; Walker & del Moral, 2008). A diferencia del N, los niveles de P y K en el suelo tienden a ser altos en los primeros estadios sucesionales debido a la meteorización de rocas ígneas y, con el paso del tiempo, tiende a disminuir su disponibilidad para las plantas debido a las pérdidas por lixiviación y a la inmovilización en sesquióxidos de Fe y Al (Walker & Syers, 1976; Veldkamp et al., 2020). Asimismo, diversos estudios han documentado la relación entre la PPN y los nutrientes edáficos (Vitousek, 1984; Cleveland et al., 2011). Por ejemplo, Vitousek (1984) y, recientemente, Cunha et al. (2022) determinaron que la disponibilidad de P, mas no la del N, limitaba la PPN de bosques. Esto, medido con la producción de hojarasca (componente de la PPN). Paoli et al. (2005) encontraron que la PPN aumenta con la disponibilidad de nutrientes. Igualmente, Cleveland et al. (2011) reportaron que la disponibilidad de P influye positivamente sobre la PPN. Todo lo anterior denota la influencia de los nutrientes sobre la PPN de bosques tropicales. Sin embargo, para probar dichas hipótesis, es necesario adicionar fertilizantes o enmiendas orgánicas (Sullivan et al., 2014), especialmente en áreas con distintos estadios sucesionales, donde los nutrientes son un factor crucial para la regeneración y la fijación de carbono.
Uno de los componentes de la PPN que es más influenciado por los nutrientes del suelo es la producción de hojarasca (Paoli & Curran 2007). Dicha producción es uno de los principales componentes de la PPN del bosque (Clark et al., 2001a; Chapin III et al., 2006) y representa más del 30 % de la PPN total en bosques tropicales (Clark et al., 2001b). Por tal razón, ha sido empleada para evaluar el efecto de la aplicación de nutrientes sobre la PPN (Sullivan et al., 2014).
En este sentido, según los experimentos que han evaluado los efectos de la fertilización en bosques tropicales, la aplicación de P y N incrementan la producción de hojarasca. Por ejemplo, Mirmanto et al. (1999) encontraron que, con la adición de N, P y NP durante cinco años, aumentó significativamente la producción de hojarasca en bosques de tierras bajas en Indonesia. Entretanto, Wright et al. (2011) reportaron un aumento en la producción de hojarasca con la aplicación de P en bosques de Panamá. Asimismo, Sayer et al. (2012) observaron una mayor producción de hojarasca con la aplicación de N, P y materia orgánica (MO), mientras que, al aplicar K y excluir la MO, disminuyó la producción. Por su parte, Kaspari et al. (2008) evidenciaron un incremento en la producción del material reproductivo de la hojarasca con la aplicación de N y P. Recientemente, Cunha et al. (2022) reportaron un incremento significativo en la producción de hojarasca con la aplicación de P en bosques amazónicos.
En síntesis, estos estudios muestran que, en bosques lluviosos tropicales maduros con suelos desarrollados, los contenidos de P y N son los principales limitantes de la producción de hojarasca, y por ende de la PPN total, no solo el P edáfico, como se planteó inicialmente (Vitousek, 1984). Contrario a ello, algunas investigaciones no han detectado cambios en la producción de hojarasca con la aplicación de nutrientes (Cusack et al., 2011, Alvarez-Clare et al., 2013). Por ejemplo, Cusack et al. (2011) no evidenciaron cambios en la hojarasca con la adición de N en bosques de Costa Rica. Asimismo, Alvarez-Clare et al. (2013) no observaron cambios en la producción de hojarasca con la aplicación de N y P. Esto quiere decir que aún hay incertidumbres y se requieren estudios para develar dicha limitación nutricional.
Adicional a lo anterior, son muy pocos los estudios que evalúan la influencia de los nutrientes sobre la PPN en bosques jóvenes de sucesiones iniciales y de reciente desarrollo edáfico (Vitousek & Farrington, 1997; Harrington et al., 2001; Campo & Vázquez-Yanes, 2004). Por ejemplo, Vitousek & Farrington (1997) probaron que, en las etapas de desarrollo edáfico, inicialmente hay limitación por N y, en etapas finales, hay limitación por P. Entretanto, Campo & Vázquez-Yanes (2004), denotaron un incremento en la producción de hojarasca con la aplicación de N y P, pero en bosques secos de sucesión temprana. Sin embargo, en bosques lluviosos tropicales de sucesión temprana, los estudios son muy pocos, y estas hipótesis de limitación de nutrientes aún no están plenamente comprobadas, por lo cual se requiere una evaluación más profunda, más aún si se tiene en cuenta que, en áreas previamente degradadas, la aplicación de nutrientes podría contribuir a la aceleración de la sucesión y la recuperación vegetal del bosque (Davidson et al., 2004, 2007). Además, en la actualidad, los bosques tropicales se encuentran fuertemente amenazados por procesos antrópicos como la ganadería, la agricultura, el aprovechamiento de madera y la minería (Valois-Cuesta & Martínez-Ruiz, 2016; Primack & Vidal, 2019; FAO & PNUMA 2020). Estas actividades son responsables de la presencia masiva de bosques secundarios de sucesión temprana (Poorter et al., 2016), en los cuales se podrían probar las hipótesis sobrela limitación nutricional de la PPN.
En Colombia, en el departamento del Chocó (Chocó biogeográfico), la minería de oro a cielo abierto genera la deforestación (Valois-Cuesta & Martínez-Ruiz, 2016) y la degradación de más de 360 ha de bosque anualmente (Ramírez & Ledezma, 2007). Desde el año 2015, se han otorgado licencias para la explotación minera de más de 302 381 ha de bosque natural, que representan el 6.49 % del territorio (Tierra Digna, 2016), con lo cual no solo se ve amenazada la biodiversidad de la región; esto también afecta la PPN y la capacidad de los ecosistemas de mitigar el cambio climático global (Quinto et al., 2013). Por tal razón, estos ecosistemas brindan una excelente oportunidad para probar las hipótesis mencionadas con anterioridad (Vitousek, 1984) en bosques de alta precipitación (8000 mm anuales) y de diferentes etapas sucesionales y de desarrollo edáfico. En consecuencia, nos planteamos las siguientes preguntas de investigación: ¿Cuáles son los efectos de la fertilización del suelo con N, P y K en la producción de hojarasca y el contenido foliar de nutrientes en bosques post-minería y bosques maduros del Chocó biogeográfico?, ¿Cuál es el nutriente más limitante de la producción de hojarasca en áreas degradadas por minería?
MATERIALES Y MÉTODOS
Área de estudio
Esta investigación se realizó en áreas boscosas previamente degradadas por minería a cielo abierto de oro en la localidad de Jigualito (5º06’01” N - 76º32’44” O), municipio de Condoto, en el Pacífico colombiano (Chocó biogeográfico). Esta región tiene una precipitación promedio de 8000 mm anuales, una altitud de 70 m y una topografía plana. Esta localidad comprende las cuencas altas del San Juan, en unidades de paisaje de piedemonte y colinas bajas con suelos húmedos de terrazas y con un tipo de roca sedimentaria transicional (Poveda et al., 2004). Los bosques son en su mayoría secundarios, con diversas edades de recuperación debido a la minería.
Los suelos son ultisoles pero, debido a la minería, presentaban mucho material rocoso y arena. Además, son ácidos y reportan altos contenidos de MO, N total, P disponible, Al y arcilla, mientras que los contenidos de Ca, K, Mg, CICE y limo, son muy bajos en áreas de reciente actividad minera. Sin embargo, su contenido es mayor en áreas con más tiempo de recuperación (Quinto et al., 2022). Por su parte, los suelos de las áreas boscosas aledañas a las minas presentan acidez extrema, con contenidos altos de Al, MO y N total y contenidos bajos de P, Mg y Ca. Asimismo, las cantidades de K son intermedias y la CICE es baja (Quinto & Moreno, 2016). En la Tabla 1 se resumen las características estructurales y edáficas de los bosques estudiados.
Tabla 1: Características estructurales, ecológicas y edáficas de los bosques maduros y bosques post-minería de 30-35 años en Jigualito, Chocó Biogeográfico, Colombia.
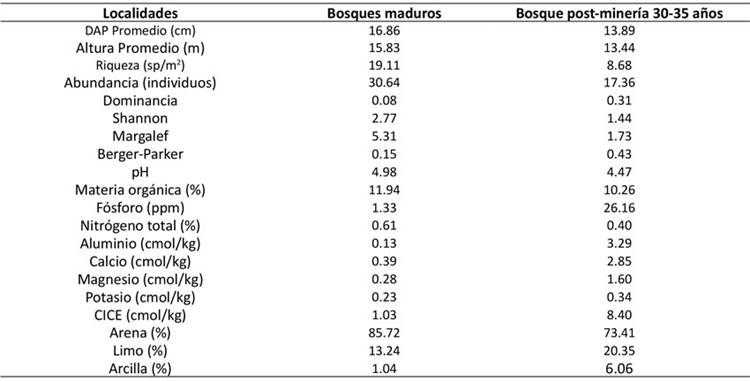
Diseño experimental
Se empleó un diseño de muestreo según el tipo de bosque y/o la edad de sucesión, con dos tipos de bosque para los muestreos. El bosque 1 correspondió a zonas que presentaban un tiempo de recuperación post-minería de 30 a 35 años. En este tipo se encontró una vegetación arbórea y arbustiva con baja diversidad y riqueza de especies (Tabla 1). El bosque 2 correspondió a bosques maduros aledaños a las minas, los cuales se tomaron como escenarios de referencia. En cada uno de los bosques se implementó un diseño factorial incompleto, con cinco tratamientos de fertilización (control, N, P, K, NPK).
Establecimiento de parcelas
En el bosque 1, correspondiente a bosques de entre 30 a 35 años post-minería, se establecieron 64 cuadrantes de 10 x 10 m (100 m2), los cuales fueron distribuidos según los tratamientos de fertilización de la siguiente manera: 16 cuadrantes de control, 12 cuadrantes para aplicar N, 12 para aplicar P, 12 para adicionar K, y 12 para fertilizar con NPK. En el bosque 2, correspondiente a bosques maduros, se emplearon tres parcelas permanentes de 100 x 100 m (1 ha) ubicadas en bosques primarios cerca de las minas abandonadas, las cuales se subdividieron en 25 cuadrantes (75 en total) de 20 x 20 m (400 m2). Estos cuadrantes fueron distribuidos según los tratamientos de fertilización de la siguiente manera: 15 cuadrantes de control, 15 cuadrantes para la aplicación de N, 15 para adicionar P, 15 para aplicar K, y 15 para fertilizar con NPK.
Aplicación de tratamientos de fertilización (N, P y K)
En cada cuadrante de muestreo experimental se aplicó un tratamiento de fertilización particular con el método de colocación al voleo (Alvarado & Raigosa, 2012). La cantidad y el número de aplicaciones por año de los fertilizantes en este estudio fueron similares a los reportados en experimentos anteriores de fertilización realizados en bosques lluviosos tropicales de baja altitud (Mirmanto et al., 1999; Wright et al., 2011; Quinto et al., 2019). Por lo tanto, la aplicación de los tratamientos se realizó durante un año, y durante este periodo se aplicarían cuatro dosis iguales de los fertilizantes en los meses de febrero, mayo, agosto y noviembre. Entretanto, la producción de hojarasca se evaluó de enero hasta diciembre. Para la aplicación de los fertilizantes, se dejó una franja de dos metros de distancia al límite de los cuadrantes, en la cual no se adicionó nutrientes. Esto, con la finalidad de evitar que los fertilizantes pasaran y contaminaran otros tratamientos diferentes. Las dosis anuales de los tratamientos fueron así. En el primer tratamiento, correspondiente a N, se aplicaron 125 kg N ha-1 año-1 en forma de urea CH4N2O, distribuidos en cuatro dosis cada año. En el segundo tratamiento, correspondiente a P, se adicionaron 50 kgP.ha-1 año-1 en forma ácido fosfórico (H3PO4), divididos en cuatro dosis anuales. En el tercer tratamiento (K) se aplicaron 50 kg K.ha-1 .año-1 en forma de cloruro de potasio (KCl), con cuatro dosis anuales. En el cuarto tratamiento, correspondiente a N+P+K, se aplicaron cada una de las dosis mencionadas en los tratamientos anteriores, y en el quinto tratamiento (control) no se aplicaron fertilizantes.
Medición de la producción de hojarasca
En cada uno de los estratos de muestreo, se instalaron colectores de hojarasca, ubicados sistemáticamente en el centro de cada cuadrante según el diseño de muestreo y los tratamientos de fertilización. En total se instalaron 139 colectores (64 colectores en bosques post-minería y 75 en bosques maduros). Los colectores fueron construidos con tubos de PVC y anjeo. Además, se fabricaron con un área de captura de 1 x 0.5 m y se instalaron a 1 m sobre el suelo. El material acumulado (hojarasca) en los colectores se recogió en bolsas plásticas para evitar pérdidas de material vegetal. La hojarasca se separó cuidadosamente en varios componentes: hojas, ramas, material reproductivo (frutos, flores y semillas), vegetación asociada y miscelánea. Esto, en aras de estimar la contribución relativa de las distintas fracciones. La información de producción de hojarasca se empleó para calcular la PPN aérea del bosque. Los muestreos de producción de hojarasca se realizaron cada quince días durante un año, y los valores de producción de hojarasca se presentaron en mega gramos por hectárea por año (Mg.ha-1.año-1).
Medición del contenido foliar de nutrientes
La hojarasca colectada por tratamiento de fertilización (control, N, P, K y NPK) en el estrato de post-minería de 30- 35 años de regeneración fue enviada al laboratorio de Biogeoquímica de la Universidad Nacional de Colombia, sede Medellín. En dicho laboratorio, se analizó el contenido de nutrientes (N, P, K, Ca, Mg) de las muestras al final del periodo de estudio mediante las técnicas específicas que se detallan en Osorio (2014) y Quinto et al. (2022). Para los bosques maduros se cuantificó el contenido foliar de nutrientes.
Análisis de datos
Para comparar la producción de hojarasca de los bosques post-minería y los bosques maduros, se empleó una prueba t-student, pues se cumplieron los supuestos de normalidad y homogeneidad de varianzas evaluadas con los estadísticos de Shapiro-Wilk y Kurtosis (entre +2,0 y -2,0). Posteriormente, para evaluar los efectos de los tratamientos de fertilización del suelo (N, P, K, NPK y control) sobre la producción de hojarasca en bosques post-minería y en bosques maduros de alta precipitación en el Chocó biogeográfico, se utilizó un análisis de varianza (ANOVA) en bosques post-minería, así como una prueba no paramétrica de Kruskal-Wallis (Kw) para los bosques maduros, dado que, en este último ecosistema, no se cumplieron los supuestos de normalidad y homogeneidad de varianzas de los datos y sus residuales (evaluados con los estadísticos de Shapiro-Wilk, Bartlett, Hartley y Kurtosis). Se empleó la prueba de rangos múltiples de Fisher en bosques post-minería y la prueba de Duncan en bosques maduros para comparar a posteriori los promedios de producción de hojarasca entre los tratamientos en los que hubo diferencias significativas con respecto al control. Finalmente, para evaluar los efectos de los tratamientos de fertilización sobre el contenido foliar de nutrientes en bosques post-minería, se utilizó un ANOVA y la prueba de rangos múltiples de Fisher. Estos análisis se realizaron a nivel general y para cada estadio sucesional. Además, se empleó el entorno de programación R (R Core Team, 2012).
RESULTADOS
La producción de hojarasca de bosques post-minería de 30 a 35 años de recuperación presentó un promedio (± desviación estándar) de 9.66 ± 1.12 Mg.ha-1.año-1, mientras que, en bosques maduros, este valor fue de 4.29 ± 0.93 Mg.ha-1.año-1. Estas diferencias en producción de hojarasca entre los dos estadios sucesionales fueron significativas (t-student=14.414; p<0.05) (Figura 1a). A nivel de tratamientos de fertilización, en bosques post-minería, la producción de hojarasca fue significativamente mayor en los tratamientos con N (11.46 Mg.ha-1.año-1), K (11.91 Mg.ha-1.año-1), y NPK (11.27 Mg.ha-1.año-1) con respecto al control (9.66 Mg.ha-1.año-1) (F4; 63=5.73; p=0.0006) (Figura 1a), por lo que se evidencia la limitación múltiple de la PPN en estos ecosistemas afectados previamente por la minería. Contrario a ello, en los bosques maduros, la producción de hojarasca fue marginalmente mayor con la aplicación de N (5.4 Mg.ha-1.año-1) con respecto al control (4.29 Mg.ha-1.año-1) (Kw4; 74=9.09; p=0.058) (Figura 1b), por lo que se evidencia la limitación de la PPN a causa de este nutriente. Igualmente, a nivel de contenidos foliares de nutrientes, en los tratamientos de fertilización solo se observó el efecto significativo de la aplicación de N sobre el contenido foliar de N (1.41 %) con respecto al control (1.21%) en la hojarasca producida en los bosques post-minería (Kw4; 74=13,1; p=0.01) (Tabla 2).
Figura 1: Efectos de la fertilización del suelo sobre la producción de hojarasca (Mg.ha-1.año-1) en bosques post-minería y maduros del Chocó Biogeográfico, Colombia. a) Bosques post-minería (F4; 63=5.73; p=0.0006), rangos múltiples de Fisher. b) Bosque maduro (Kruskal-Wallis4; 74=9.09; p=0.058), rangos múltiples de Duncan. Las letras (a y b) denotan diferencias estadísticamente significativas.
Tabla 2: Efectos de la fertilización (control, N, P, K, NPK) del suelo sobre los contenidos foliares de nutrientes de la hojarasca de bosques post-minería del Chocó Biogeográfico. Las letras (a y b) significan diferencias significativas. Los asteriscos denotan la significancia de los p-valores estadísticos: *0.05.

Figura 1. Efectos de la fertilización del suelo sobre la producción de hojarasca (Mg.ha-1.año-1) en bosques post-minería y maduros del Chocó Biogeográfico, Colombia. a) Bosques post-minería (F4; 63=5.73; p=0.0006), rangos múltiples de Fisher. b) Bosque maduro (Kruskal-Wallis4; 74=9.09; p=0.058), rangos múltiples de Duncan. Las letras (a y b) denotan diferencias estadísticamente significativas.
DISCUSIÓN
¿Cuál es la producción de hojarasca de las áreas post-minería en bosques del Chocó biogeográfico en comparación con los bosques naturales?
En este estudio, la producción de hojarasca fue de 9.66 Mg.ha-1.año-1 en bosques secundarios de entre 30 y 35 años post-minería en el Chocó biogeográfico, valores que se encuentran dentro del rango de producción de bosques lluviosos tropicales, i.e., entre 5.2 y 12.5 Mg.ha-1.año-1 (Chave et al., 2010). Asimismo, esta producción de bosques secundarios fue superior a la registrada en los bosques primarios aledaños a las minas (4.29 Mg.ha-1.año-1) y a las observadas previamente en bosques maduros con suelos infértiles de la región del Chocó biogeográfico, con 7.82, 7.35, 8.1 y 7.2 Mg.ha-1.año-1 en las localidades de Opogodó, Pacurita, Bajo Calima y Salero respectivamente (Rodríguez, 1989, Quinto et al., 2017). Esto podría ser evidencia de una mayor producción de hojarasca en bosques secundarios tropicales en comparación con bosques maduros y primarios. Esta tendencia es similar a lo reportado por Loumeto (2003), quien observó una mayor producción de hojarasca en los bosques secundarios del Congo (10.7 Mg.ha-1.año-1) en comparación con los primarios (6.1 Mg.ha-1.año-1) tropicales. Esto también fue similar a lo observado por Barlow et al. (2007), quienes reportaron una mayor producción de hojarasca (13.4 Mg.ha-1.año-1) en un bosque secundario de la Amazonía brasileña. Igualmente, Ostertag et al. (2008), en bosques sucesionales de Puerto Rico, evidenciaron una mayor producción de hojarasca en bosques secundarios de 60 años de recuperación. Esto, en comparación con la producción de los bosques primarios en el primer año de medición.
Sin embargo, los resultados de este estudio fueron contrarios a lo evidenciado por Dantas & Phillipson (1989), Moreno & Oberbauer (2008) y Chave et al. (2010), quienes observaron mayores tasas de producción de hojarasca en bosques primarios tropicales en comparación con los secundarios. En efecto, en términos sucesionales, algunas investigaciones han reportado que la producción de hojarasca puede incrementar con el tiempo de sucesión vegetal, con edades de entre 1.5 y 28 años (Brown & Lugo 1990). No obstante, otras investigaciones no presentan dichas tendencias (Barlow et al., 2007; Ostertag et al., 2008). Algunos estudios incluso muestran que, en etapas sucesionales superiores a los 60 años, la producción puede ser similar o incluso superior a la de un bosque primario (Ostertag et al., 2008), como se evidenció en este estudio. En síntesis, las diferentes tendencias en la producción de hojarasca con la sucesión o edad de regeneración muestran que este componente de la PPN puede estar determinado en mayor proporción por otras variables como el tipo de bosque, el tipo de suelo, la estacionalidad de la precipitación (Chave et al., 2010), el viento, la temperatura, la radiación solar (Ferreira et al., 2014; Zhu et al., 2022), la composición florística de especies dominantes en bosques secundarios y plantaciones (Celentano et al., 2011), los niveles de pluviosidad (Schuur, 2003), nutrientes como N total y MO (Quinto et al., 2017), el contenido de P edáfico y foliar (Cleveland et al., 2011) y la disponibilidad de K, Ca, Mg, Fe, así como P disponible, N total y P total (Paoli & Curran, 2007). Basado en lo anterior, se puede deducir que la mayor producción de hojarasca registrada en los bosques secundarios post-minería posiblemente se deba a la influencia de la mayor disponibilidad de minerales (P disponible, Ca, Mg y CICE) (Tabla 1) generados por la meteorización de la roca provocada por la minería (Quinto et al., 2022). Esto es similar a lo observado en estudios realizados previamente en bosques tropicales, donde se registró una mayor producción de hojarasca sobre suelos más fértiles y con mayor disponibilidad de nutrientes (Vitousek & Sanford, 1986; Paoli & Curran, 2007; Quinto et al., 2017), y evidencia un mayor reciclaje de MO y minerales como mecanismo natural para recuperar el suelo, mantener el funcionamiento del ecosistema y restaurar la PPN total del bosque luego de los disturbios generados por la minería a cielo abierto, que son comunes en la región del Chocó biogeográfico.
¿Cuáles son los efectos de la fertilización del suelo con N, P y K sobre la producción de hojarasca en bosques post-minería y bosques maduros del Chocó biogeográfico?
En bosques post-minería, la producción de hojarasca fue mayor con la aplicación de N, K y NPK, lo denota una limitación múltiple de la PPN aérea en estos ecosistemas afectados por la minería a cielo abierto. Estos resultados corroboran parcialmente la hipótesis de que, en suelos tropicales de etapas sucesionales iniciales, hay una limitación de la PPN por el N edáfico (Walker, 1993; Walker & del Moral, 2008), pues se evidenció que la producción de hojarasca estuvo limitada por múltiples nutrientes (N, K y N+P+K) en estas áreas post-minería, no solo por el N del suelo. Igualmente, lo evidenciado en bosques post-minería fue similar a lo reportado experimentalmente por Harrington et al. (2001), quienes observaron una mayor PPN aérea y una mayor producción de hojarasca con la aplicación de N y N+P en bosques secundarios de suelos recién formados en Hawaii. Asimismo, estos resultados son similares a lo reportado por Campo & Vázquez-Yanes (2004), quienes evidenciaron un incremento en la producción de hojarasca con la aplicación de N+P, pero en bosques secos tropicales de sucesión temprana. En síntesis, estos estudios muestran que la PPN aérea de bosques secundarios tropicales con suelos en desarrollo inicial -o, en este caso, áreas degradadas por minería- está limitada principalmente por la disponibilidad de N edáfico, ya sea solo o aplicado concomitantemente con otros minerales como K o P (Harrington et al., 2001; Campo & Vázquez-Yanes, 2004), o P+K, como se evidenció en este estudio.
El hecho de que exista una limitación de la PPN aérea por el N edáfico en bosques post-minería denota que, efectivamente, la minería a cielo abierto genera daños significativos en el ecosistema, que afectan la dinámica y el reciclaje de nutrientes del suelo, en este caso el N edáfico y, con ello, la PPN total y el balance del carbono. Asimismo, esta limitación de la producción de hojarasca por N edáfico puede ser causada por distintas razones que afectan el bosque de manera conjunta. Primero, luego de la minería, los suelos quedan alterados y con condiciones muy similares a etapas sucesionales iniciales (Quinto et al., 2022). Las plantas y bacterias con capacidad de fijar simbióticamente el N2 atmosférico comienzan a colonizar estos ambientes (Walker 1993; Sansupa et al., 2021), generando limitación por N edáfico. Un ejemplo de ello fue reportado por Sansupa et al. (2021), quienes mostraron que, con la minería a cielo abierto, se reduce la actividad, la abundancia, la funcionalidad (descomposición, mineralización, amonificación y nitrificación), la riqueza y la biomasa microbial del suelo post-minería y, con ello, se promueve la limitación de la PPN por N edáfico.
Segundo, aunque en los bosques post-minería hay presencia de especies arbóreas con capacidad de fijar simbióticamente N atmosférico (Fabaceae) y el contenido edáfico de N total es relativamente alto (N total=0.40 %) (Tabla 1), los altos niveles de precipitación (>8000 mm anuales) de la región y la textura arenosa de los suelos post-minería facilitan conjuntamente la lixiviación de nutrientes (Quinto & Moreno, 2016), no solo el N fijado, sino también minerales como K y P edáficos. Este fenómeno podría explicar la limitación múltiple (N, K y N+P+K) de la PPN aérea de estos bosques afectados.
Tercero, aunque los contenidos de nutrientes como N, P, Ca y K son altos, se presentan también altos contenidos de y toxicidad por Al (Quinto et al., 2022), lo cual afecta la asimilación por parte de las plantas (Chandra & Keshavkant, 2021). En este sentido, se ha demostrado que los altos niveles de Al edáfico disminuyen la elongación de raíces, reducen el crecimiento del tallo, alteran procesos metabólicos y fisiológicos y disminuyen la PPN del bosque (Shetty et al., 2021). Asimismo, el Al edáfico reduce la absorción de nutrientes, produce estrés nutricional, clorosis y necrosis, y disminuye el tamaño de hojas y su fotosíntesis (Chandra & Keshavkant, 2021). En consecuencia, se puede afirmar que la toxicidad por Al también podría ser responsable de la limitación múltiple de la producción de hojarasca. Así, la limitación múltiple de nutrientes (N, K y N+P+K) en bosques post-minería del Chocó biogeográfico puede ser el resultado de la acción conjunta de la poca colonización de plantas y bacterias fijadoras de nitrógeno, la lixiviación de nutrientes generada por la alta precipitación y la toxicidad por Al que limita la absorción de nutrientes del suelo. Todo lo anterior afecta conjuntamente la producción de hojarasca, y por ende de la PPN aérea.
Otro aspecto importante a destacar en las áreas post-minería es la evidencia de la limitación de la PPN por el contenido edáfico de K, el cual ha sido determinado experimentalmente como un nutriente limitante de la PPN en bosques tropicales (Wright et al., 2011; Sayer et al., 2012). Particularmente, Tripler et al. (2006) concluyeron, en un meta-análisis sobre la dinámica del K en bosques, que este macronutriente limita el funcionamiento (incluida la PPN) de bosques tropicales y subtropicales, conclusiones se corroboran experimentalmente en este estudio. En síntesis, este es el primer estudio que confirma experimentalmente la limitación de la PPN aérea por la aplicación de K en bosques tropicales secundarios post-minería. Además, se evidencia la poca limitación que tiene el P edáfico en estos ambientes sobre la PPN aérea, posiblemente debido a su alta disponibilidad, generada por la meteorización de rocas ígneas (Walker & Syers, 1976; Veldkamp et al., 2020), y a la acción mecánica de la minería sobre la textura del suelo (Quinto et al., 2022). Estos factores, en conjunto, aumentan la disponibilidad del mineral para las plantas.
Por su parte, en los bosques maduros, la producción de hojarasca fue mayor con la aplicación de N, lo que evidencia la limitación nutricional de la PPN aérea por este mineral. Estos resultados son similares a lo planteado en la ‘ley del mínimo’, según la cual “el crecimiento de una planta depende de la cantidad de alimento que se le presenta en cantidades mínimas” (Salisbury & Ross, 1994) , como se evidencia en la producción de hojarasca con la aplicación de N en bosques maduros. Esto es similar a lo reportado por LeBauer & Treseder (2008), que en un meta-análisis reportaron la influencia del N del suelo sobre la PPN de bosques tropicales. Asimismo, estos resultados, en los que se evidencia la influencia de un nutriente como principal limitante de la PPN del bosque, son similares a las recientes observaciones de Cunha et al. (2022), quienes demostraron experimentalmente la limitación del P edáfico respecto a la producción de hojarasca, la producción de raíces finas y la PPN total de bosques maduros tropicales de la Amazonía. Ambas investigaciones -Cunha et al. (2022) y este estudio- muestran que la PPN aérea en bosques tropicales puede estar limitada por la disponibilidad de un nutriente (N o P) (Vitousek, 1984; LeBauer & Treseder, 2008; Cunha et al., 2022) según la ley del mínimo (Salisbury & Ross, 1994). Además, es importante mencionar que la mayoría de las investigaciones han considerado al P edáfico como el principal limitante de la PPN de bosques lluviosos tropicales de baja altitud (Vitousek, 1984; Cleveland et al., 2011; Cunha et al., 2022). Sin embargo, esta investigación muestra lo contrario, i.e., una mayor limitación de la PPN aérea por el N del suelo.
La limitación de la producción de hojarasca por el N del suelo posiblemente se deba a la significativa influencia que tiene este mineral sobre la fotosíntesis y el crecimiento de los árboles (Salisbury & Ross, 1994; Lamber et al., 2008; Quinto & Moreno, 2022). Asimismo, nuestros resultados muestran que, aunque estos ecosistemas poseen altos contenidos de N total (Quinto & Moreno, 2016) y ostentan altas tasas de fijación simbiótica de N (Cleveland et al., 1999), los bajos contenidos de otros minerales como P disponible, Ca, y Mg pueden limitar la fijación biológica de N atmosférico (Vitousek et al., 2013), generando así una limitación por el N en el ecosistema boscoso. En consecuencia, es lógico afirmar que hay una posible co-limitación (P y N) de la PPN aérea en bosques lluviosos tropicales de baja altitud.
Al respecto, algunas investigaciones muestran que, con la aplicación concomitante de N y P, se produce una mayor PPN en el ecosistema.
Por ejemplo, Mirmanto et al. (1999) encontraron que, con la adición de N, P y NP durante cinco años, se incrementó significativamente la producción de hojarasca en bosques de tierras bajas en Indonesia. Asimismo, Harrington et al. (2001) observaron experimentalmente una mayor PPN aérea con la aplicación de P y N+P en bosques maduros de suelos viejos desarrollados de Hawaii. Igualmente, Campo & Vázquez-Yanes (2004) constataron una mayor producción de hojarasca con la aplicación conjunta de N+P en bosques secos tropicales maduros de la península de Yucatán, en México. Por su parte, Wright et al. (2011) reportaron un aumento en la producción de hojarasca con la aplicación de P, mientras que Sayer et al. (2012) observaron una mayor producción de hojarasca con la aplicación de N, P y MO. Asimismo, Kaspari et al. (2008) evidenciaron un incremento en la producción de material reproductivo de la hojarasca con la aplicación de N y P en bosques de Panamá.
En síntesis, estos estudios evidencian la influencia de la aplicación de N y/o P sobre la producción de hojarasca según el tipo de bosque, el tipo de suelos y la disponibilidad de otros nutrientes (Wright et al., 2018), lo que denota una limitación de la PPN aérea, no solo por el P, como se planteó hace unas décadas (Vitousek, 1984), sino también por el nutriente que realmente limite el ecosistema tropical estudiado, como se evidenció en este estudio.
¿Cuáles son los efectos de la fertilización del suelo con N, P y K sobre el contenido foliar de nutrientes en bosques post-minería en el Chocó biogeográfico?
En bosques post-minería, el contenido foliar de N fue mayor con la aplicación de N, lo que denota una limitación nutricional por dicho nutriente. Esta limitación posiblemente se deba a la acción conjunta de la poca colonización de plantas y bacterias fijadoras de N atmosférico (Sansupa et al., 2021), la lixiviación del N debido a la alta precipitación, la textura arenosa de las áreas post-minería (Quinto et al., 2022) y la toxicidad por Al que limita la absorción de N y otros minerales del suelo (Chandra & Keshavkant, 2021; Shetty et al., 2021). Esto no solo afecta la producción de hojarasca, sino también los contenidos foliares de N y, con ello, el reciclaje de nutrientes.
Estudios de hace pocos años muestran que los contenidos foliares de nutrientes pueden ser utilizados como variables ecológicas para evaluar la limitación del funcionamiento y la PPN de ecosistemas boscosos (Cleveland et al., 2011; Sullivan et al., 2014; Wright et al., 2018). Por ejemplo, Cleveland et al. (2011) evidenciaron correlaciones entre los contenidos foliares de P y los contenidos edáficos de P total en bosques tropicales, los que, a su vez, presentaron mayor correlación con la PPN aérea. Asimismo, en bosques secundarios de suelos recién formados de Hawaii, se evidenció que la aplicación de N y P incrementa los contenidos foliares de estos elementos (Harrington et al., 2001). En este mismo sentido, se comprobó experimentalmente que la adición de N, P y N+P aumentó los contenidos foliares de P en bosques secos secundarios en México (Campo et al., 2007). Igualmente, en los experimentos factoriales realizados en Panamá, se corroboró que, con la aplicación de N, P y K, se generó un incremento en la concentración foliar de cada nutriente aplicado según el tratamiento (Wright et al., 2018). Estos resultados, en conjunto, indican que la aplicación de nutrientes devela la limitación no solo de la PPN del bosque, sino también del nivel nutricional del ecosistema estudiado, como se observó en áreas post-minería.
Acknowledgements
AGRADECIMIENTOS
Esta investigación fue financiada mediante el proyecto Evaluación del efecto de la fertilización del suelo sobre la producción neta del ecosistema en áreas degradadas por minería, como estrategia para potenciar la captura de carbono y la venta de servicios ambientales en el Chocó Biogeográfico (código 1128-852-72243) de la Universidad Tecnológica del Chocó DLC, la Universidad Nacional de Colombia, sede Medellín, la Universidad de Valladolid (España), el Instituto de Investigaciones Ambientales del Pacífico John Von Neumann y el SENA. Dicho proyecto fue aprobado por Ministerio de Ciencia, Tecnología e Innovación.
REFERENCIAS
Licencia
Derechos de autor 2024 Colombia forestal

Esta obra está bajo una licencia internacional Creative Commons Atribución-CompartirIgual 4.0.
Colombia Forestal conserva los derechos patrimoniales (copyright) de las obras publicadas, y favorece y permite la reutilización de las mismas bajo la licencia Creative Commons Atribución-CompartirIgual 4.0 Internacional por lo cual se pueden copiar, usar, difundir, transmitir y exponer públicamente, siempre que:
Se reconozcan los créditos de la obra de la manera especificada por el autor o el licenciante (pero no de una manera que sugiera que tiene su apoyo o que apoyan el uso que hace de su obra).